Addiction Circuitry in the Human Brain
Abstract
A major challenge in understanding substance-use disorders lies in uncovering why some individuals become addicted when exposed to drugs, whereas others do not. Although genetic, developmental, and environmental factors are recognized as major contributors to a person’s risk of becoming addicted, the neurobiological processes that underlie this vulnerability are still poorly understood. Imaging studies suggest that individual variations in key dopamine-modulated brain circuits, including circuits involved in reward, memory, executive function, and motivation, contribute to some of the differences in addiction vulnerability. A better understanding of the main circuits affected by chronic drug use and the influence of social stressors, developmental trajectories, and genetic background on these circuits is bound to lead to a better understanding of addiction and to more effective strategies for the prevention and treatment of substance-use disorders.
Reprinted with permission from the Annual Review of Pharmacology and Toxicology 2012; 52:321–336
Introduction
The rewarding effect of drugs of abuse is the main reason why humans use them and laboratory animals self-administer them (1). However, most individuals using drugs never escalate to uncontrollable levels of drug use and only a relatively small percentage does (approximately 10–20%). These are the individuals who fit diagnostic criteria (as per DSM IV) for substance abuse or dependence (compulsive drug use with loss of control over intake despite adverse consequences) (2). Who becomes addicted (term used in lieu of the dependence term used by DSM IV to avoid confusion with physical dependence) and who does not are strongly influenced by genetic (50% of risk), developmental (risk is higher in adolescence than adulthood), and environmental factors (drug access, stress), as well as the type of drug (some drugs produce addiction faster than others, e.g., methamphetamine versus marijuana) (2).
It is generally accepted that the rewarding effects of drugs are due to their ability to increase dopamine (DA) particularly in the nucleus accumbens (NAc) (3). The potency of drugs as well as the mechanism by which they increase DA differs for the various drug classes (4). Interestingly, DA’s role in drug reward (as well as reward in general) does not seem to equate with hedonic pleasure (mediated in part by endogenous opioids and cannabinoids), but instead DA appears to encode prediction of reward, imprinting incentive value to reinforcers (energizing approach behavior) and facilitating learning of reward associations (conditioning) through its modulation of subcortical (including the NAc) and cortical brain regions (reviewed in Reference 5).
Initial work on DA’s role in drug reward focused on the mesolimbic DA pathway—DA neurons in the ventral tegmental area (VTA) projecting into the NAc (1). More recent work now recognizes the crucial role of the mesostriatal (projections from substantia nigra into dorsal striatum) and mesocortical pathways (projections from the VTA into the frontal cortex) in drug reward and addiction (1). DA signals through five different receptors of which the most ubiquitous are the D1 (D1R) and D2 receptors (D2R), which are also the ones that are best understood. The low-affinity D1R is stimulated by large DA increases as induced by phasic DA cell firing and is implicated in drug reward and conditioning. The high-affinity D2R is stimulated by relatively low DA concentrations, as are induced by tonic DA cell firing, and it may interfere with drug reward (6). Differential adaptation in D1R versus D2R signaling pathways with repeated drug administration is likely to underlie neuroplastic changes in addiction. Overall in animal studies, the increases in D1R signaling are associated with sensitized responses to drugs, and the decreases in D2R signaling with compulsive drug intake (7, 8). Neuroplastic changes in D1R- and D2R-modulated pathways are driven in part by modification in the expression of AMPA and NMDA receptors in glutamatergic synapses (9).
Neurotransmitters other than DA (e.g., cannabinoids, opioids) are also involved with drug reward, and their relative contribution to a drug’s rewarding effects is determined in part by their pharmacological effects (e.g., endogenous opioids for heroin, alcohol, nicotine). Similarly, several neurotransmitters are also involved in the neuroadaptations associated with addiction (e.g., decreases in endogenous opioid signaling in cocaine addiction), including the central role of glutamate in the neuroplastic changes associated with chronic drug exposures (10, 11).
Clinical studies have used positron emission tomography (PET) to evaluate DA’s role in drug reward and addiction (and, to a lesser extent, the role of endogenous opioids), whereas fMRI studies have concentrated on delineating the neuronal pathways affected by drugs in addicted subjects. These findings show that addiction affects not only the reward circuit, but also circuits involved with memory (conditioning/habits), motivation (energy, drive), executive function (inhibitory control, salience attribution, decision making), mood (stress reactivity and hedonic state), and interoception (internal awareness).This review focuses on the findings derived from brain-imaging studies with special attention to studies targeting DA pathways because these have been most widely investigated.
Drug Reward in Addiction
In humans, several drugs [stimulants (12, 13), nicotine (14), alcohol (15, 16) and marijuana (17)] increase DA in the dorsal and ventral striatum. These studies are based on the measurement of DA D2 receptors that are not occupied by DA (receptor availability) and thus able to bind the PET radiotracers (i.e., [11C]raclopride, [18F]fallypride, or [11C]PHNO). By comparing D2/3 receptor availability after placebo and after acute drug exposures, these studies estimate the relative changes in DA (18). Most drugs tested in humans have shown a significant increase in DA in the ventral striatum (where the NAc is located) that was associated with the subjective perception of the drugs as rewarding (reviewed in Reference 19).
In addition, PET studies have shown that the pharmacokinetic profiles of drugs are crucial for their reinforcing effects. In particular, the speed at which drugs enter the human brain is associated with its rewarding effects. By labeling various drugs with positron emitters, it has been possible to monitor their pharmacokinetics in the human brain. These studies have shown that, after intravenous administration, nicotine reaches peak levels in 2–3 min, cocaine in 4–6 min, and methamphetamine in 10–15 min. In addition, at least for stimulant drugs, the fast drug uptake is associated with the “high” described by drug users (Figure 1) (20, 21). The various routes of drug administration (oral, smoked, injected) influence the speed at which drugs are delivered into the brain, which in turn affects their rewarding effects. For example, when cocaine enters the brain rapidly (smoked or intravenous administration) it elicits a more intense “high” than when it enters the brain more slowly (snorted) even when the cocaine doses given to participants are adjusted to produce the same levels of DA transporter blockade in the brain, which is the mechanism by which cocaine increases DA and produces reward (22). The dependence of drugs’ rewarding effects on their ability to induce “fast” and large DA increases probably reflects the fact that it mimics the fast and large DA increases associated with phasic DA firing that are associated in the brain with conveying information about reward and saliency (23).
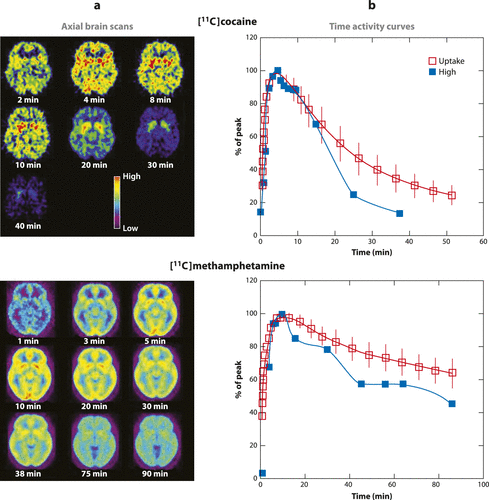
Figure 1. Pharmacokinetics of Cocaine and Methamphetamine in the Human Brain and Relationship to the Drug-Induced “High.” (a) Axial Brain Images of the Distribution of [11C]Cocaine and [11C]Methamphetamine at Different Times (Minutes) after their Administration. (b) Time Activity Curves for the Concentration of [11C]Cocaine and [11C]Methamphetamine in Striatum Alongside the Temporal Course for the “High” Experienced after Intravenous Administration of these Drugs. Modified from References 20 and 21. Note that the Fast Brain Uptake of these Drugs Corresponds with the Temporal Course of the “High,” Which Suggests that the “High” is Associated with the “Rate of Dopamine Increases.”*
* A full-color version of the figure, as originally published, appears in the online version of this article (focus.psychiatryonline.org).
Functional MRI (fMRI) is a noninvasive technique that allows us to monitor changes in brain physiology that are based on the increase in arterial blood flow to the local vasculature that accompanies neural activation. Because oxygenated hemoglobin (arterial blood) produces a different magnetic signal than that produced by deoxygenated hemoglobin (venous blood), this is the basis of the BOLD (blood-oxygen-level dependence) contrast used to track changes in regional brain activity at rest or upon stimulation. Imaging studies with fMRI to assess the activation/deactivation responses of the human brain to acute drug administration have reported that in cocaine abusers acute administration of cocaine induced deactivation of the ventral striatum (negative BOLD) that correlated with the high (24). In addition, such studies also reported a negative correlation with BOLD signals in the inferior frontal and orbitofrontal cortex as well as the anterior cingulated cortex (ACC), whereas craving correlated positively with activity in these regions. It is interesting to note that most fMRI studies evaluating the effects of stimulant drugs on reward report a deactivation of BOLD signals in the NAc rather than BOLD increases as may have been expected by activation of this brain region. In contrast, increases in BOLD are associated with craving (25). The deactivation of the NAc by cocaine is consistent with studies in nonhuman primates that also show inhibition of the NAc with cocaine administration, which were interpreted to reflect the predominance of D2R (inhibit cyclic AMP) over D1R (increase cyclic AMP) in the NAc (26). One important aspect to consider in fMRI studies assessing the effects of acute cocaine is that for ethical reasons these cannot be done in non-drug-abusing controls. Thus, it is not possible to determine if the BOLD responses in the NAc reflect the pharmacological effects of cocaine or, alternatively, the adaptations associated with repeated drug use that in turn alter subsequent responses to the drug. This is because stimuli (including drugs) that induce fast and large DA increases also trigger conditioned responses, as a result of which the conditioned stimuli can increase DA (27).
Indeed, through conditioning, a neutral stimulus that is linked with the reinforcer (in this case, the drug) acquires the ability—on its own—to increase DA in the NAc in anticipation of the reward, and preclinical studies have shown that this effect is associated with drug-seeking behaviors (27). When exposed to a conditioned stimulus, the DA neurons in animals trained to expect a natural reinforcer (e.g., food) stop responding to the primary reinforcer and instead respond to the conditioned stimulus (23). These studies have also shown that, when the reward is expected and does not occur, DA cell firing is inhibited and DA release in the NAc is decreased. Thus, at this time, we cannot rule out the possibility that the decreases in BOLD detected through fMRI studies in the NAc of cocaine abusers after intravenous cocaine administration could reflect a decreased deactivation as a response to a drug whose effects are not as intense as may have been expected.
To answer this question, studies have compared the ability of stimulant drugs [methylphenidate (MPH) and amphetamine (AMPH)] to increase DA in the striatum of addicted versus nonaddicted subjects. Similar to cocaine, MPH increases DA by blocking DA transporters, whereas AMPH (similar to methamphetamine) increases DA by releasing it from the terminal using the DA transporter as carrier. Intravenous injection of these stimulant drugs is highly rewarding, and cocaine abusers report the effects to be similar to those of intravenous cocaine. Cocaine-addicted but detoxified subjects show marked attenuation of DA increases in the striatum induced by MPH or AMPH (at least 50% lower) and an attenuation of their self-reported “high,” when compared with non-drug-abusing controls (20, 28). Moreover, a more recent study provides the first comparison of responses to intravenous MPH in actively using cocaine abusers and showed that in these subjects MPH effects on striatal DA could not be distinguished from placebo (29). A comparison with the responses of the non-drug-abusing controls revealed the striatal DA changes in the cocaine abusers were 80% lower than in controls (Figure 2). Marked blunting of striatal DA increases to MPH and to AMPH have also been reported in detoxified alcoholics (reviewed in Reference 19).
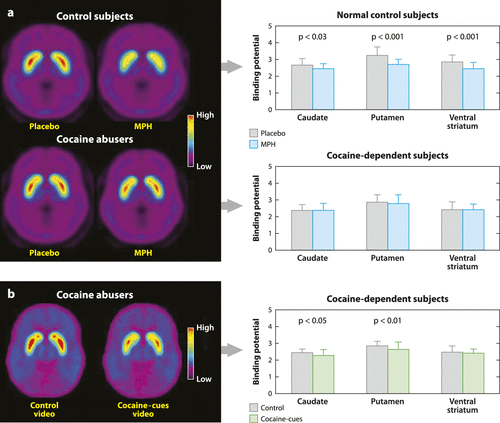
Figure 2. Dopamine (DA) Changes Induced by Intravenous Methylphenidate (MPH) in Controls and in Active Cocaine Abusers and by Cocaine Cues in Active Cocaine Abusers. (a) Average Nondisplaceable Binding Potential (BPND) Images of [11C]Raclopride in Active Cocaine-Addicted Subjects and Controls Tested after Placebo and after Intravenous MPH. MPH Reduced D2R Availability in Controls but not in Cocaine-Addicted Subjects. Note that Cocaine Abusers Show both Decreases in Baseline Striatal D2R Availability (Placebo Measure) and Decreases in DA Release when given Intravenous MPH (Measured as Decreases in D2R Availability from Baseline). (b) Average BPND Images of [11C]Raclopride in Cocaine Abusers Tested while viewing a Neutral Video (Nature Scenes) and while viewing a Cocaine-Cues Video (Subjects Administering Cocaine). The Cocaine Cues Decreased D2R in Caudate and Putamen. Modified from Reference 32. Note that Cocaine-Addicted Subjects did not Respond to Intravenous MPH but Instead Responded to the Cocaine-Cues Exposure.*
* A full-color version of the figure, as originally published, appears in the online version of this article (focus.psychiatryonline.org).
The attenuated (or abolished) DA increases in response to stimulant drugs in cocaine addiction is paradoxical because cocaine-induced DA increases are linked to its rewarding effects, a finding that had led to the hypothesis of a sensitized response to the DA-enhancing effects of drugs (enhanced reward) that drove the exaggerated motivation to procure the drug in addiction. Similarly, the deactivation of the NAc after intravenous cocaine use suggests that these responses are adaptations that follow repeated drug administration (some related to tolerance but others to conditioning) rather than being a result of only the pharmacological effects of the drug.
Conditioning in Addiction
Conditioning is one of the initial neuroadaptations that follows exposure to drugs and involves DA phasic signaling (predominantly though activation of D1R) and synaptic changes in NMDA and AMPA receptors (30, 31). These conditioned responses are believed to underlie the intense desire for the drug that occurs when addicted subjects are exposed to drug cues and that triggers their compulsive use of the drug.
Brain imaging studies to assess DA responses to conditioned cues have shown that active cocaine abusers exhibit significant DA increases in the striatum when they are exposed to videos depicting scenes of individuals procuring and administering cocaine. In addition, the magnitude of this increase was correlated with the subjective experience of craving (32, 33). Cocaine abusers with the largest cue-induced DA increases in the dorsal striatum also scored higher on addiction severity, thereby suggesting that a basic disruption in addiction may be enhanced reactivity of DA mesostriatal pathways to conditioned responses. The dorsal striatum is implicated in habit learning; thus, its engagement with cue exposures is likely to reflect the strengthening of habits as chronicity of addiction progresses.
Thus, in active cocaine abusers, the DA increases triggered by conditioned cues appear to be larger than those produced by intravenous MPH. Moreover, the DA increases triggered by the cues are associated with a desire for the drug, which suggests that conditioned responses may drive the DA signaling that triggers and maintains the motivation to take the drug. Indeed, fMRI studies of exposure to drug cues have consistently shown activation of the NAc with exposure to drug cues, which contrasts with studies in which subjects were administered cocaine and investigators reported decreases in BOLD signals in the NAc (25).
Nonetheless, regardless of the extent to which the drug (even when its DA-enhancing effects are attenuated) predicts reward, the act of its administration (injection, smoke, drink) becomes a conditioned cue so that the mere expectation of the reward during administration of the drug may increase DA in the NAc. On the basis of these findings and given the role of DA in predicting reward, we postulate a shift in the source of DA increases: Although drugs may initially lead to DA release in the NAc (i.e., signaling reward), with repeated administration and as habits develop, the DA increases become transferred from the pharmacological effects of the drug to the conditioned responses (i.e., to the behaviors and objects linked with administering the drugs, such as the act of injecting or seeing a syringe). Such an effect is reported to occur also with repeated exposure to natural reinforcers (23).
Brain imaging studies have shown that cue-elicited craving is associated not only with increased activation of the NAc (34–36), but also with activation of other reward regions including the VTA, orbitofrontal cortex, insula, and ACC (37–40). This suggests that processing of conditioned responses in the brain (as well as processing of reward) is the result of functional networks that include cortical and subcortical brain regions rather than involvement of isolated brain regional effects. Indeed, preclinical studies have revealed that glutamatergic projections from the prefrontal cortex (PFC) into the VTA/SN, NAc, and dorsal striatum as well as projections from the amygdala to the NAc are involved in these conditioned responses (10).
Executive Function and Corticostriatal Circuits in Addiction
Imaging studies have been providing consistent evidence for the involvement of the PFC in the addiction process (41). In parallel, preclinical and clinical research has expanded our understanding of the complex role that the various regions in the PFC play in cognition. These identify a prominent role for the PFC in executive function that includes inhibitory control, decision making, emotional regulation, purposefulness, motivation, and salience attribution, among others. This explains why PFC disruption in addiction can have such catastrophic consequences across a wide range of behaviors (34).
The PFC works in tandem with striatal regions via corticostriatal networks that are modulated by DA (42). Imaging studies have documented disruption of these complex pathways, which have been investigated predominantly for the D2R-mediated signaling pathways and to a limited extent for the D1R-signaling pathway. In addicted subjects (to various drug classes including cocaine, heroin, alcohol, methamphetamine, nicotine, cannabinoids), a consistent finding reported from PET studies and corroborated by preclinical studies of chronic drug administration is that of significant reductions in D2R availability in the striatum, which persists months after detoxification (reviewed in Reference 19). Brain imaging studies on the effects of chronic drugs on D1R are few, and results are not always consistent. Specifically, in studies with nonhuman primates that were chronically exposed to cocaine, some investigators reported increases in D1R (43, 44), whereas others reported decreases (45). The conflicting reports suggest that the availability of D1R could be very sensitive to the characteristics (e.g., dosage, duration, frequency, and abstinent periods) of cocaine administration (46). In humans, imaging (PET) studies of cocaine abusers reported no changes in D1R (46), and postmortem studies of cocaine abusers and alcoholics reported no changes in D1R protein or mRNA (47).
Brain imaging studies have shown that reductions in striatal D2R in addicted subjects are associated with decreases in the activity of several prefrontal regions (Figure 3). These include the orbitofrontal cortex (involved in salience attribution and goal-directed behaviors), ACC (involved in inhibitory control and awareness), and the dorsolateral PFC (involved in higher cognitive operations and decision making) (35, 36, 48). Thus, improper DA modulation of these prefrontal regions in addicted subjects could underlie the enhanced incentive motivational value of drugs and the users’ loss of control over drug intake (41). Because impairments in the orbitofrontal cortex and ACC are associated with compulsive behaviors and impulsivity, we also postulate that DA’s impaired modulation of these regions could underlie the compulsive and impulsive drug taking in addiction (49, 50). Indeed, preclinical and clinical studies have shown that low striatal D2R levels are associated with impulsivity (51, 52), and in rodents, impulsivity predicts compulsive cocaine administration. In turn, overexpression of D2R in the striatum interferes with compulsive alcohol (53) and cocaine (54) intake. Imaging studies have also documented that in addicted subjects there is a decrease in DA release in the striatal regions (55) that is likely to further exacerbate an already low signaling from the reduced striatal D2R levels, which exert an inhibitory role on drug reward (56).
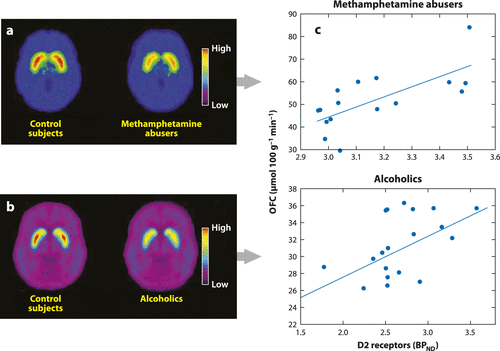
Figure 3. Brain Dopamine D2 Receptors (D2R) in Controls and in Methamphetamine Abusers and Alcoholics and Association between D2R in the Striatum and Metabolism in the Orbitofrontal Cortex. (a) Average Brain Images for D2R Availability (BPND) in Control Subjects and in Methamphetamine Abusers Obtained with [11C]Raclopride. (b) Average Brain Images for D2R Availability (BPND) in Control Subjects and in Alcoholics Obtained with [11C]Raclopride. (c) Correlations between Striatal D2R and Metabolism in the Orbitofrontal Cortex (OFC) in Methamphetamine Abusers (top) and in Alcoholics (bottom). Modified from Reference 19.*
* A full-color version of the figure, as originally published, appears in the online version of this article (focus.psychiatryonline.org).
The fundamental role of the PFC in addiction is evidenced by the increased recognition that impairments in executive function may increase the vulnerability for a substance-use disorder. Thus, it is possible that in some addicted subjects the underlying vulnerability to drugs was triggered by impairments in PFC function and that subsequent repeated drug use led to decreases in striatal D2R. Evidence for this comes from a study of subjects at high risk for alcoholism: These subjects, who were not alcoholics but had a family history of alcoholism, exhibited a higher than normal striatal D2R availability that was associated with normal metabolism in the orbitofrontal cortex, ACC, and dorsolateral PFC (34). We interpreted this to suggest that the upregulation of striatal D2R allowed these high-risk individuals to maintain adequate prefrontal function, which protected them against alcohol abuse.
Impaired self-control plays a fundamental role in drug-taking behaviors in addiction. Successful self-regulation functions requires top-down control from the PFC to the striatal and limbic regions involved with rewards and emotions (reviewed in Reference 57). Impaired self-control in addicted subjects is believed to reflect disrupted prefrontal regulation of striatal regions. The level of impairment is influenced by the emotional state (negative mood increases impairment) and the context (exposure to unexpected cues can also impair it). Indeed, in cocaine abusers, cognitive control of craving is associated with decreased activation of the NAc and the orbitofrontal cortex, which is dependent on proper activity of the inferior PFC (BA 44) (58) (Figure 4). Moreover, lateral PFC activity assessed in cigarette smokers performing an inhibitory task (an experimental proxy of the self-control needed against temptations or distractions) predicted their craving and smoking behavior over the subsequent three-week period (59).
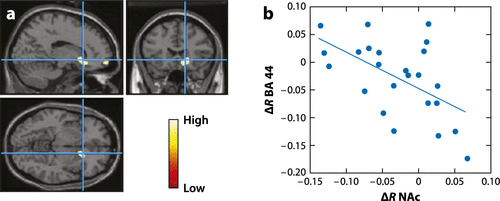
Figure 4. (a) Areas of the Brain where Metabolism Differed when Cocaine Abusers were Exposed to the Cocaine-Cues Video with a Directive to Purposefully Inhibit Craving Versus when they were Exposed to the Cocaine-Cues Video with no Inhibition. Metabolism was Significantly Lower in the right NAc and in the right Orbitofrontal Cortex with Inhibition than it was without Inhibition (Statistical Parametric Mapping Results p < 0.005 not Corrected, Cluster > 100 Voxels). (b) Regression Slope for the Differences in Metabolism (Inhibition Versus no Inhibition) in the right NAc and in the right BA 44 (r = −0.55, p < 0.006).*
* A full-color version of the figure, as originally published, appears in the online version of this article (focus.psychiatryonline.org).
Disrupted executive function is also implicated in the exaggerated bias toward immediate smaller rewards at the expense of larger delayed ones that occurs in addiction (60). Specifically, damage of the orbitofrontal cortex in humans (61) and in rodents (62) increases the tendency to choose immediate rewards over larger delayed rewards. This is likely to contribute to addicted subjects’ tendency to choose the immediate gratification expected from taking the drug over any greater but delayed gratification that could be derived from staying sober.
Damage to the orbitofrontal cortex also interferes with the inhibition of responding to formerly rewarding cues that are no longer reinforcing, thus favoring the emergence of perseverative behaviors even when these are no longer reinforcing (reviewed in Reference 63). Thus, the dysregulated activity of the orbitofrontal cortex could underlie both the impulsive choices for immediate rewards and compulsive drug intake even when the drug-induced DA increases may be profoundly attenuated in addicted subjects (as described above).
Motivation for Drug Reinforcers in Addiction
DA plays a critical role in motivation (e.g., vigor, persistence, effort, behavioral activation) through its regulation of several target regions including the NAc, ACC, orbitofrontal cortex, dorsolateral PFC, amygdala, dorsal striatum, and ventral pallidum. Specifically, DA is implicated in the motivation costs required to complete tasks that demand high levels of effort (64). Mesolimbic DA signaling may energize goal seeking and influence cost-benefit evaluation in behavioral decisions (65).
The enhanced motivation to procure drugs is a hallmark of addiction, and this is well captured on the “incentive salience” hypothesis of addiction. This hypothesis proposes that, in addiction, drugs acquire enhanced “incentive salience” whereupon the drugs are consumed, not because they are liked (hedonic value), but because they are “wanted” (incentive value of reward) (66). In fact, the decrease in subjective responses to drugs along with the reduced drug-induced increases in DA support the notion that the motivation to procure drugs is not driven by drug “liking” but rather by an enhanced motivational drive to procure them. Indeed, drug-addicted individuals will go to extreme behaviors to obtain drugs even at the expense of seriously adverse consequences (2). In an addicted person, drug seeking and drug taking become the main motivational drives displacing all other activities (67). As a result, the addicted person is aroused and motivated when seeking to procure the drug and lacks motivation to pursue non-drug-related activities.
Brain imaging studies have monitored the shifts in regional brain activity of addicted persons when exposed to drug-conditioned cues versus when exposed to non-drug-related cues. When studied without exposure to drug stimuli, substance abusers showed significant decreases in activity of prefrontal regions implicated in motivation including the orbitofrontal cortex and ACC (reviewed in Reference 19). In contrast, these prefrontal regions become activated when drug abusers were exposed to drug-related stimuli (39, 68–70). Similarly, cocaine abusers studied shortly after an episode of cocaine binging showed increased metabolic activity in the orbitofrontal cortex and ACC (as well as the dorsal striatum), which was associated with drug craving (71).
The enhanced sensitivity of the motivational circuitry to drugs in addicted subjects is exemplified by a study by Volkow et al. (72): Whereas cocaine-addicted subjects exposed to a stimulant drug showed increased metabolism in the ventral ACC and medial orbitofrontal cortex (an effect that was associated with craving for the administered drug), nonaddicted subjects exposed to the same drug had decreased metabolism in these regions. This suggests that activation of these prefrontal regions with drug exposure may be specific to addiction and associated with the enhanced desire for the drug.
The modulation of motivation by drug cues in addiction may occur even outside of conscious awareness. Indeed, in cocaine abusers, exposure to subliminal cocaine cues induced activation of striatal and limbic brain regions (73). Moreover, these activation patterns predicted the affective responses to stimuli that were subsequently presented to subjects in a way that was visible to them. Overall, these imaging findings corroborate the involvement of the orbitofrontal cortex, ACC, and striatum (including the NAc) in the enhanced motivational value of drug stimuli in addiction and provide evidence for the involvement of unconscious processes in shaping motivation for drugs.
Mood and Stress Reactivity in Addiction
Negative emotions including those triggered by exposure to stressful stimuli provide an important prompt in drug taking. This may reflect the adverse effects that negative emotions have on self-control (57). Indeed, an imaging study in cocaine abusers assessed the effects of distressing stimuli and showed that such stimuli decreased activity in prefrontal regions while increasing it in striatal regions (74). In these subjects, the activation of striatal regions was associated with stress-induced cocaine craving and is consistent with a decrease in top-down control upon exposure to stressful stimuli.
The neurobiological mechanisms underlying the enhanced reactivity to negative emotions including those triggered by stressful stimuli have not been thoroughly investigated in human subjects. Preclinical studies suggest that these involve the CRF and noradrenergic systems that modulate stress reactivity (75) as well as an upregulated kappa opioid receptor signaling in the brain (76). Recent studies also suggest that the lateral habenula may be involved in drug relapse in addiction (77), including relapses triggered by negative emotionality and stress. The lateral habenula is neuroanatomically connected to circuits involved with reward and emotion (78). In nonhuman primates, neurons in the lateral habenula are inhibited by reward-predicting stimuli and activated by aversive-predicting stimuli or by unrewarded trials (79). Given that activation of the lateral habenula inhibits DA neurons in the VTA and the substantia nigra (79), this could be a mechanism that triggers drug use to compensate for the reduced DA signaling following DA-induced cell inhibition.
The lateral habenula also projects to the median and dorsal raphe nuclei, which contain serotonin neurons and regulate emotions (80). In addition, electrical stimulation of the lateral habenula inhibits serotonin neurons (81), which is consistent with a role for this anatomical structure in depression and anxiety (82). Thus, the lateral habenula, which is activated by aversive stimuli (including stress) (83) and linked with negative emotions and whose activation is further linked with drug relapse could be involved in mechanisms underlying the high relapse rate linked with negative mood and/or stress exposures. There are no reported imaging studies on the habenula in addiction, which is likely to reflect in part the small size of this brain region, which makes it difficult to image.
Self-Awareness in Addiction
In addiction, there is also evidence of a significant disruption in self-awareness, which includes the ability to recognize internal mental states (emotions, desires, and personal representation of one’s abilities). This is likely to impair an addicted individual’s awareness of disease, his/her need for treatment, and/or his/her strong desire for the drug (84). The neurocircuitry underlying self-awareness is not completely understood, but the default mode network (DMN) has been implicated in this process (85). The DMN includes regions from the dorsal and ventral ACC, insula, precuneus, and parietal cortex that become deactivated when performing a task but are active when mind-wandering (86, 87). Regions from the DMN that have been directly associated with impaired awareness in addiction include both the ACC and the insula.
Summary
Several brain circuits are relevant in the neurobiology of addiction and result in an enhanced motivational value of the drug (secondary to learned associations through conditioning and habits) at the expense of other reinforcers (secondary to decreased sensitivity of the reward circuit) and an impaired ability to inhibit the intentional actions associated with the strong desires to take the drug (secondary to impaired executive function) that result in compulsive drug taking in addiction (67). In addition, disrupted executive function contributes to impaired insight in addiction, which interferes with the recognition of disease and the need for treatment. Because these neuronal systems also play fundamental roles in social behaviors (88), understanding their disruption in addiction is providing insight into why drugs can be so disruptive to social relationships and why the typical positive reinforcers used to promote positive behaviors (e.g., pay increases, promotion) or the typical negative reinforcers used to deter negative behaviors (e.g., incarceration, fines) are by themselves ineffective in stopping drug use in addicted subjects (89). This model suggests a multipronged therapeutic approach to addiction designed to strengthen the neuronal systems that become disrupted in addiction.
1 . 2009. Roles for nigrostriatal—not just mesocorticolimbic—dopamine in reward and addiction. Trends Neurosci. 32:517–24Crossref, Google Scholar
2 . 2005. The neuroscience of addiction. Nat. Neurosci. 8:1429–30Crossref, Google Scholar
3 . 1988. Drugs abused by humans preferentially increase synaptic dopamine concentrations in the mesolimbic system of freely moving rats. Proc. Natl. Acad. Sci. USA 85:5274–78Crossref, Google Scholar
4 . 2005. Is there a common molecular pathway for addiction? Nat. Neurosci. 8:1445–49Crossref, Google Scholar
5 . 2010. Brain reward circuitry beyond the mesolimbic dopamine system: a neurobiological theory. Neurosci. Biobehav. Rev. 35:129–50Crossref, Google Scholar
6 . 2009. D2R striatopallidal neurons inhibit both locomotor and drug reward processes. Nat. Neurosci. 12:393–95Crossref, Google Scholar
7 . 2008. Opposing patterns of signaling activation in dopamine D1 and D2 receptor-expressing striatal neurons in response to cocaine and haloperidol. J. Neurosci. 28:5671–85Crossref, Google Scholar
8 . 2011. Repeated cocaine exposure decreases dopamine D2-like receptor modulation of Ca2+ homeostasis in rat nucleus accumbens neurons. Synapse 65:168–80Crossref, Google Scholar
9 . 2008. Region-specific changes in the subcellular distribution of AMPA receptor GluR1 subunit in the rat ventral tegmental area after acute or chronic morphine administration. J. Neurosci. 28:9670–81Crossref, Google Scholar
10 . 2009. The glutamate homeostasis hypothesis of addiction. Nat. Rev. Neurosci. 10:561–72Crossref, Google Scholar
11 . 1992. Neural mechanisms of drug reinforcement. Ann. N.Y. Acad. Sci. 654:171–91Crossref, Google Scholar
12 . 1999. Reinforcing effects of psychostimulants in humans are associated with increases in brain dopamine and occupancy of D2 receptors. J. Pharmacol. Exp. Ther. 291:409–15Google Scholar
13 . 2001. Amphetamine-induced dopamine release in human ventral striatum correlates with euphoria. Biol. Psychiatry 49:81–96Crossref, Google Scholar
14 . 2009. Ventral striatal dopamine release in response to smoking a regular versus a denicotinized cigarette. Neuropsychopharmacology 34:282–89Crossref, Google Scholar
15 . 2003. Alcohol promotes dopamine release in the human nucleus accumbens. Synapse 49:226–31Crossref, Google Scholar
16 . 2010. A genetic determinant of the striatal dopamine response to alcohol in men. Mol. Psychiatry 16:809–17Crossref, Google Scholar
17 . 2009. Δ9-tetrahydrocannabinol induces dopamine release in the human striatum. Neuropsychopharmacology 34:759–66Crossref, Google Scholar
18 . 1997. Schizophrenia is associated with elevated amphetamine-induced synaptic dopamine concentrations: evidence from a novel positron emission tomography method. Proc. Natl. Acad. Sci. USA 94:2569–74Crossref, Google Scholar
19 . 2009. Imaging dopamine’s role in drug abuse and addiction. Neuropharmacology 56(Suppl. 1):3–8Crossref, Google Scholar
20 . 1995. Is methylphenidate like cocaine? Studies on their pharmacokinetics and distribution in the human brain. Arch. Gen. Psychiatry 52:456–63Crossref, Google Scholar
21 . 2008. Fast uptake and long-lasting binding of methamphetamine in the human brain: comparison with cocaine. Neuroimage 43:756–63Crossref, Google Scholar
22 . 2000. Effects of route of administration on cocaine induced dopamine transporter blockade in the human brain. Life Sci. 67:1507–15Crossref, Google Scholar
23 . 2010. Dopamine signals for reward value and risk: basic and recent data. Behav. Brain Funct. 6:24Crossref, Google Scholar
24 . 2005. Neural responses to acute cocaine administration in the human brain detected by fMRI. Neuroimage 28:904–14Crossref, Google Scholar
25 . 2005. Neural correlates of high and craving during cocaine self-administration using BOLD fMRI. Neuroimage 26:1097–1108Crossref, Google Scholar
26 2011. fMRI of cocaine self-administration in macaques reveals functional inhibition of basal ganglia. Neuropsychopharmacology 36:1187–98Crossref, Google Scholar
27 . 2009. Neural encoding of cocaine-seeking behavior is coincident with phasic dopamine release in the accumbens core and shell. Eur. J. Neurosci. 30:1117–27Crossref, Google Scholar
28 . 2007. Amphetamine-induced dopamine release: markedly blunted in cocaine dependence and predictive of the choice to self-administer cocaine. Am. J. Psychiatry 164:622–29Link, Google Scholar
29 . 2010. Decreased brain dopaminergic responses in active cocaine dependent subjects. J. Nucl. Med. 51(Suppl. 2):269Crossref, Google Scholar
30 . 2009. Disruption of NMDAR-dependent burst firing by dopamine neurons provides selective assessment of phasic dopamine-dependent behavior. Proc. Natl. Acad. Sci. USA 106:7281–88Crossref, Google Scholar
31 . 2007. Synaptic plasticity and addiction. Nat. Rev. Neurosci. 8:844–58Crossref, Google Scholar
32 . 2006. Cocaine cues and dopamine in dorsal striatum: mechanism of craving in cocaine addiction. J. Neurosci. 26:6583–88Crossref, Google Scholar
33 . 2006. Increased occupancy of dopamine receptors in human striatum during cue-elicited cocaine craving. Neuropsychopharmacology 31:2716–27Crossref, Google Scholar
34 . 2006. High levels of dopamine D2 receptors in unaffected members of alcoholic families: possible protective factors. Arch. Gen. Psychiatry 63:999–1008Crossref, Google Scholar
35 . 1993. Decreased dopamine D2 receptor availability is associated with reduced frontal metabolism in cocaine abusers. Synapse 14:169–77Crossref, Google Scholar
36 . 2001. Low level of brain dopamine D2 receptors in methamphetamine abusers: association with metabolism in the orbitofrontal cortex. Am. J. Psychiatry 158:2015–21Crossref, Google Scholar
37 . 2000. Cue-induced cocaine craving: neuroanatomical specificity for drug users and drug stimuli. Am. J. Psychiatry 157:1789–98Crossref, Google Scholar
38 . 1999. Functional imaging of craving. Alcohol Res. Health 23:187–96Google Scholar
39 . 1999. Regional brain metabolic activation during craving elicited by recall of previous drug experiences. Life Sci. 64:775–84Crossref, Google Scholar
40 . 1999. Limbic activation during cue-induced cocaine craving. Am. J. Psychiatry 156:11–18Crossref, Google Scholar
41 . 2000. Addiction, a disease of compulsion and drive: involvement of the orbitofrontal cortex. Cereb. Cortex 10:318–25Crossref, Google Scholar
42 . 2003. The primate basal ganglia: parallel and integrative networks. J. Chem. Neuroanat. 26:317–30Crossref, Google Scholar
43 . 2009. Abstinence from chronic cocaine self-administration alters striatal dopamine systems in rhesus monkeys. Neuropsychopharmacology 34:1162–71Crossref, Google Scholar
44 . 2002. Effects of cocaine self-administration on striatal dopamine systems in rhesus monkeys: initial and chronic exposure. Neuropsychopharmacology 27:35–46Crossref, Google Scholar
45 . 1998. Effect of cocaine self-administration on dopamine D2 receptors in rhesus monkeys. Synapse 30:88–96Crossref, Google Scholar
46 . 2009. Dopamine D1 receptors in cocaine dependence measured with PET and the choice to self-administer cocaine. Neuropsychopharmacology 34:1774–82Crossref, Google Scholar
47 . 2005. Striatal dopamine D1 receptors in type 1 and 2 alcoholics measured with human whole hemisphere autoradiography. Brain Res. 1031:20–29Crossref, Google Scholar
48 . 2007. Profound decreases in dopamine release in striatum in detoxified alcoholics: possible orbitofrontal involvement. J. Neurosci. 27:12700–6Crossref, Google Scholar
49 . 1996. Cocaine addiction: hypothesis derived from imaging studies with PET. J. Addict. Dis. 15:55–71Crossref, Google Scholar
50 . 2002. Drug addiction and its underlying neurobiological basis: neuroimaging evidence for the involvement of the frontal cortex. Am. J. Psychiatry 159:1642–52Crossref, Google Scholar
51 . 2009. Striatal dopamine D2/D3 receptor availability is reduced in methamphetamine dependence and is linked to impulsivity. J. Neurosci. 29:14734–40Crossref, Google Scholar
52 . 2008. Review. Neural mechanisms underlying the vulnerability to develop compulsive drug-seeking habits and addiction. Philos. Trans. R. Soc. Lond. B 363:3125–35Crossref, Google Scholar
53 . 2001. Overexpression of dopamine D2 receptors reduces alcohol self-administration. J. Neurochem. 78:1094–1103Crossref, Google Scholar
54 . 2008. D2R DNA transfer into the nucleus accumbens attenuates cocaine self-administration in rats. Synapse 62:481–86Crossref, Google Scholar
55 . 2011. Addiction: beyond dopamine reward circuitry. Proc. Natl. Acad. Sci. USA. 108:15037–42Crossref, Google Scholar
56 . 2011. Addiction: Decreased reward sensitivity and increased expectation sensitivity conspire to overwhelm the brain’s control circuit. BioEssays 32:748–55Crossref, Google Scholar
57 . 2011. Cognitive neuroscience of self-regulation failure. Trends Cogn. Sci. 15:132–39Crossref, Google Scholar
58 . 2009. Cognitive control of drug craving inhibits brain reward regions in cocaine abusers. Neuroimage 49:2536–43Crossref, Google Scholar
59 . 2011. In the trenches of real-world self-control: neural correlates of breaking the link between craving and smoking. Psychol. Sci. 22:498–506Crossref, Google Scholar
60 . 2006. A review of delay-discounting research with humans: relations to drug use and gambling. Behav. Pharmacol. 17:651–67Crossref, Google Scholar
61 . 2004. Impulsivity, time perception, emotion and reinforcement sensitivity in patients with orbitofrontal cortex lesions. Brain 127:1108–26Crossref, Google Scholar
62 . 2002. Effects of lesions of the orbitofrontal cortex on sensitivity to delayed and probabilistic reinforcement. Psychopharmacology 160:290–98Crossref, Google Scholar
63 . 2007. Reconciling the roles of orbitofrontal cortex in reversal learning and the encoding of outcome expectancies. Ann. N. Y. Acad. Sci. 1121:320–35Crossref, Google Scholar
64 . 2007. Effort-related functions of nucleus accumbens dopamine and associated forebrain circuits. Psychopharmacology 191:461–82Crossref, Google Scholar
65 . 2011. Dopamine signaling in the nucleus accumbens of animals self-administering drugs of abuse. Curr. Top. Behav. Neurosci. 3:29–71Crossref, Google Scholar
66 . 2007. The debate over dopamine’s role in reward: the case for incentive salience. Psychopharmacology 191:391–431Crossref, Google Scholar
67 . 2003. The addicted human brain: insights from imaging studies. J. Clin. Investig. 111:1444–51Crossref, Google Scholar
68 . 1999. Association of methylphenidate-induced craving with changes in right striato-orbitofrontal metabolism in cocaine abusers: implications in addiction. Am. J. Psychiatry 156:19–26Crossref, Google Scholar
69 . 1996. Activation of memory circuits during cue-elicited cocaine craving. Proc. Natl. Acad. Sci. USA 93:12040–45Crossref, Google Scholar
70 . 2003. Brain imaging studies in human addicts. Eur. Neuropsychopharmacol. 13:453–58Crossref, Google Scholar
71 . 1991. Changes in brain glucose metabolism in cocaine dependence and withdrawal. Am. J. Psychiatry 148:621–26Crossref, Google Scholar
72 . 2005. Activation of orbital and medial prefrontal cortex bymethylphenidate in cocaine-addicted subjects but not in controls: relevance to addiction. J. Neurosci. 25:3932–39Crossref, Google Scholar
73 . 2008. Prelude to passion: limbic activation by “unseen” drug and sexual cues. PLoS One 3:e1506Crossref, Google Scholar
74 . 2005. Neural activity associated with stress-induced cocaine craving: a functional magnetic resonance imaging study. Psychopharmacology 183:171–80Crossref, Google Scholar
75 . 2001. A role for the CRF-containing pathway from central nucleus of the amygdala to bed nucleus of the stria terminalis in the stress-induced reinstatement of cocaine seeking in rats. Psychopharmacology 158:360–65Crossref, Google Scholar
76 . 2009. kappa-Opioid receptor signaling and brain reward function. Brain Res. Rev. 62:127–46Crossref, Google Scholar
77 . 2011. Identification of brain nuclei implicated in cocaine-primed reinstatement of conditioned place preference: a behaviour dissociable from sensitization. PLoS One 5:e15889Crossref, Google Scholar
78 . 2008. Habenula: crossroad between the basal ganglia and the limbic system. J. Neurosci. 28:11825–29Crossref, Google Scholar
79 . 2007. Lateral habenula as a source of negative reward signals in dopamine neurons. Nature 447:1111–15Crossref, Google Scholar
80 . 1979. Efferent connections of the habenular nuclei in the rat. J. Comp. Neurol. 187:19–47Crossref, Google Scholar
81 . 1977. Physiological evidence for habenula as major link between forebrain and midbrain raphe. Science 197:89–91Crossref, Google Scholar
82 . 1982. The dorsal diencephalic conduction system: a review of the anatomy and functions of the habenular complex. Neurosci. Biobehav. Rev. 6:1–13Crossref, Google Scholar
83 . 2008. The lateral habenula: no longer neglected. CNS Spectr. 13:484–89Crossref, Google Scholar
84 . 2009. The neurocircuitry of impaired insight in drug addiction. Trends Cogn. Sci. 13:372–80Crossref, Google Scholar
85 . 2008. The brain’s default network: anatomy, function, and relevance to disease. Ann. N. Y. Acad. Sci. 1124:1–38Crossref, Google Scholar
86 . 2007. A default mode of brain function: a brief history of an evolving idea. Neuroimage 37:1083–90; discussion 1097–99Crossref, Google Scholar
87 . 2007. Wandering minds: the default network and stimulus-independent thought. Science 315:393–95Crossref, Google Scholar
88 . 2007. Social decision-making: insights from game theory and neuroscience. Science 318:598–602Crossref, Google Scholar
89 . 2009. Treating drug abuse and addiction in the criminal justice system: improving public health and safety. JAMA 301:183–90Crossref, Google Scholar